Spinal cord protection for thoracoabdominal aortic aneurysm repair: from bench to bedside
Introduction
Ischemia of the spinal cord and subsequent injury remains a devastating complication in aortic surgery. Spinal cord injury (SCI) manifests across a broad spectrum both sub-clinically at a histologic level in the laboratory setting, or clinically as lower extremity paraparesis or paralysis, from temporary motor deficits to complete paralysis (Figure 1).
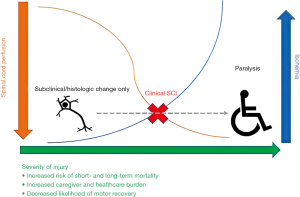
SCI takes a massive toll on patients with increased morbidity, short and long-term mortality and decreased quality of life, as well as vastly increasing healthcare costs (2,3). Furthermore, SCI places a heavy burden on caregivers, with a large proportion of SCI caregivers reporting moderate to severe care burden levels (4). Clinical experience has led to many known risk factors for SCI, including numerous comorbidities, surgical acuity, extension and complexity of aortic operations and destruction of collateral flow (2,5,6) (a further description of risk factors is outlined in Table 1).
Table 1
Preoperative |
Male, non-white race, increasing age |
Higher ASA class |
Hypertension |
COPD |
Any renal disease |
Anemia |
History of smoking |
Any CAD |
Prior ligation or occlusion of collateral sources (e.g., subclavian, iliac arteries) |
Surgical acuity |
Dissection and associated malperfusion |
Intraoperative |
Adjunct procedures |
Extent of segmental artery sacrifice |
Extent of aortic replacement or coverage |
Distal landing zone 5–10 |
Loss of collateral flow from subclavian/iliac arteries |
Duration of aortic cross-clamping |
Hypotension, hypoxia, anemia |
Postoperative |
Hypotension, hypoxia, anemia |
ASA, American Society of Anesthesiologists; COPD, chronic obstructive pulmonary disease; CAD, coronary artery disease.
SCI occurs typically through ischemic insult in watershed areas as a result of changes in spinal cord blood flow (SCBF), whether it be intraoperatively related to extensive period of aortic clamping, post-operatively related to the sacrifice of essential blood vessels such as the thoracic, intercostal or lumbar segmental arteries (SA), or through a combination of the above mechanisms. Furthermore, after perfusion is restored and sufficient sources of perfusion remain, the subsequent inflammatory response because of reperfusion causes a significant secondary insult. In some settings, embolic ischemic injury can play a role as well, especially in the case of thoracic endovascular aortic repair (TEVAR) with the subsequent dislodgement of aortic plaque related to wire manipulation and device deployment. In cases of embolic injury, perfusion is usually irrecoverable. However, the vast majority are related to the above low flow states and ischemia-reperfusion injury (5).
Although we have made great strides in understanding and managing SCI, paraplegia persists as a significant complication. In this article, we review the perfusion of the spinal cord and the pathophysiology of SCI, the basic and clinical science surrounding collateralization and ischemia-reperfusion injury, and other clinical adjuncts used in the prevention of SCI.
Anatomy of spinal cord perfusion
Numerous SA contribute to arterial blood flow to the spinal cord, which originate from branches of the subclavian (vertebral, ascending cervical, deep cervical), the intercostal, the lumbar and the lateral sacral arteries (arising from the internal iliac arteries) (Figure 2). The anterior spinal artery courses along the spinal cord, merging with the SA from the other branches of the aorta, and in turn giving off sulcal arteries that supply the ventral two-thirds of the spinal cord.
Paired posterior spinal arteries arising from the vertebral arteries, or from the posterior inferior cerebellar artery, course medially to the posterior root entry zone and supply the dorsal third of the cord, including the dorsal horn and dorsal columns. The vasocorona, a tightly organized vessel network, connects these two sources of supply, and sends branches into the white matter around the margin of the spinal cord. Among the reinforcing segmental medullary arteries, the artery of Adamkiewicz, or the great anterior segmental medullary artery, arises typically at the level of T8-L1 and provides a significant proportion of flow to this region of the spine (Figure 3). Previously, preventing spinal cord ischemia was focused on preserving this artery, but as we have gained more understanding of spinal cord perfusion, more emphasis is placed on the broader collateral network.
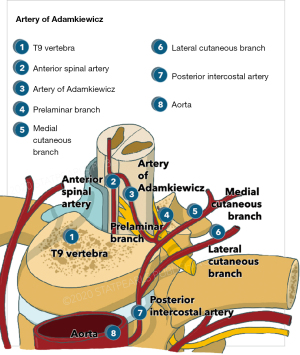
Collateralization
The previous thought that SCI was driven by severance of the artery of Adamkiewicz was primarily driven by a 1993 report that SCI was as high as 30% when the entire thoracoabdominal aorta was resected (8). The work by Dr. Griepp provided new insight into the anatomy and physiology of spinal cord perfusion by identifying the vast network of collaterals that are responsible. Their work with porcine models demonstrated rich interconnections between the anterior spinal artery and adjacent back musculature, as well as the connections between vasculature at certain levels of the spinal cord and the levels above and below it. Furthermore, they found connections to the subclavian and hypogastric arteries, and to the internal thoracic artery via the intercostal arteries (9).
This robust network of collateralization can greatly compensate for even complete destruction of SA. Studies have demonstrated that spinal cord perfusion pressure (SCPP) is approximately 80% of mean arterial pressure, and with destruction of SA falls to 25% (9). However, post-operatively, SCPP returns to baseline within five days regardless of the presence of SCI. Staged artery sacrifice lessens the drop in SCPP to only 50–70% with significant decreases in SCI (10). Given the above, collateralization has been an important focus of basic and clinical research as a means to combat SCI. The ultimate clinical goal is to ensure sufficient collateralization exists for watershed areas to prevent SCI, and to provide adequate support until natural collateralization occurs and recovers baseline perfusion.
At the bench: collateralization & cellular mechanisms of angiogenesis
The cellular concepts surrounding collateralization remains an important area of investigation, with a specific focus on angiogenesis and the development of new collateralization in either the pre-operative setting to better tolerate destruction of perfusion, or post-operatively to treat insufficient perfusion. A number of important mechanisms have been identified, including the phosphoinositide 3 kinase (PI3K)/antiapoptotic kinase (AKT)/endothelial nitric oxide synthase (eNOS) pathway, the extracellular signal-regulated kinase 1 and 2 (ERK 1/2)/vascular endothelial growth factor (VEGF) pathway, the Jag/Notch and the Midkine regulatory cytokine signaling cascade (11). Manipulating these complex networks remains difficult given the interplay between pathways, and that responses of various agonists and antagonists that act on these systems can either benefit or harm angiogenesis depending on dosing and timing during the ischemic period. Hopefully, in time, research into these pathways will provide both diagnostic information through biomarkers, and possible pharmacologic interventions that promote angiogenesis.
Clinical application of collateralization
Although the mechanisms behind collateralization and pharmacologic targets remain an ongoing area of investigation, it is already applied clinically and remains an ongoing area of clinical investigation. There has been some work looking at possible modalities of using collateralization to obtain diagnostic information about risk of SCI. Namely, collateral network near-infrared spectroscopy has been proposed as a minimally invasive method to measure spinal cord perfusion, and has been used in porcine models to accurately measure tissue perfusion, however, this has not been applied to human subjects (12).
Collateralization is applied in surgical planning to reduce the ischemic burden at any one time in more extensive repairs, and thus allowing for less perfusion burden at once. In practice, this is primarily done by two means: through staged means maintaining pre-existing collateralization and allowing for new collaterals to form, or by directly promoting new collateralization.
The most common method used is a serial approach to more extensive anatomic reconstructions. For example, if a proximal TEVAR can augment an extent II to an extent III repair, or if an extent II or III can be augmented to an extent IV, this reduces the initial ischemic burden, and allows for development of a robust collateral network. Additionally, given in many situations the quality of native tissue is poor and difficult to suture, the presence of a TEVAR provides an added buttress for anastomosis. The development of a collateral network is further facilitated by optimizing inflow revascularization, such as through an iliofemoral or subclavian bypass prior to repair. Although the definition of staging varies amount centers, numerous studies have demonstrated efficacy, and it is for most centers considered the standard of care.
Another proposed staging method is temporary aneurysm sac perfusion (TASP). The theory behind TASP is that inducing an endoleak and allowing temporary perfusion of an aneurysm sac will prevent complete aneurysm sac thrombosis. This in turn will maintain pre-existing collateral networks or allow new ones to form. Following that, the remaining branch vessel graft is performed, thus completing the aneurysm exclusion. TASP has shown benefit when compared with single-stage repair; a study of 83 patients who underwent branched endovascular aortic repair (EVAR) with or without TASP demonstrated a significantly lower rate of SCI with TASP (13). However, this practice has not been investigated in US centers, owing to two primary reasons; the first being that it has not been compared with conventional means of staged repair, and the second is the theoretical risks with TASP, namely coagulopathy related to high flow endoleaks, and the risk of rupture (14).
SA embolization or sacrifice has been proposed as means to directly promote collateralization. The idea behind this method is that sacrifice will allow for “arteriogenic preconditioning”, development of collateral perfusion networks and thus maintenance of SCPP. In porcine models, significantly better motor outcomes and preserved SCPP has been seen in staged SA sacrifice (10). A retrospective analysis of 90 open cases found that staged sacrifice resulted in significantly lower rates of SCI (0% vs. 15%) (15), and a more recent European cohort of 57 patients who underwent endovascular embolization had no SCI at 30 days following definitive TEVAR repair (16). This method has not been adopted by any US aortic center to date, however, these initially promising results have prompted the MIS2ACE trial; a European randomized trial that is ongoing for SA coil embolization.
Ischemia-reperfusion injury
Both open and endovascular interventions result in some degree of regional cord ischemia. Although we tend to view SCI as a binary outcome, this likely underestimates the prevalence of ischemic injury. Murine models have demonstrated histologically that even with preserved motor function, significant cytoarchitectural changes and neuronal degeneration occur, with the severity of microscopic changes correlating with motor changes (17).
As a whole, inadequate SCBF leads to an imbalance between spinal cord metabolic demand and oxygen supply. This leads to a consumption of cells’ adenosine triphosphate (ATP) stores after the cessation of neuronal oxidative phosphorylation. ATP-dependent sodium/potassium pumps are unable to function, and thus cells are unable to maintain a normal electrochemical gradient (18). This results in a build of sodium within the cell, resulting in membrane depolarization and osmotic swelling. Consequently, voltage-sensitive calcium channels are activated, driving massive calcium influx. Additionally, glutamate is released activating N-methyl-D-aspartate (NMDA) and α-amino-3-hydroxy-5-methyl-4-isoxazolepropionic acid (AMPA) receptor dependent ion channels, which further increase intracellular calcium. This phenomenon results in the cascading response known as excitotoxicity, which is thought to further exacerbate cell damage, and may represent the ultimate cause of neuronal demise (18). Following neuronal apoptosis, inflammatory mediators are released, recruiting microglia and neutrophils. The buildup of inflammatory cytokines is thought to occur in a bimodal distribution. The initial wave of inflammation is likely driven by damaged neurons and activated local inflammatory cells, damaging surrounding tissue and endothelium. This leads to tissue swelling, further diminishing SCPP and producing a secondary ischemic insult. This secondary wave leads to further inflammatory mediator release, and in murine models correlated with delayed motor dysfunction (19). It is clear that SCI is not just limited to initial ischemic insult, but also to metabolic intolerance driven by excitotoxic, inflammatory and oxidative processes. Inducing metabolic tolerance and preserving neuronal viability has become a focal point of SCI research, however, given the numerous complex cellular processes involved, it has remained a scientific and clinical challenge.
At the bench: promoting tolerance to ischemia-reperfusion injury
Currently, there are no pharmacologic agents for the induction of metabolic tolerance to spinal cord ischemia-reperfusion injury for complex aortic intervention. The idea behind inducing metabolic tolerance is predicated upon the notion that brief periods of ischemia results in an increased tolerance to a more significant ischemic insult. In animal models this has been well established; short periods of ischemia improve local SCBF, spinal cord tissue oxygenation and motor outcomes (20). This has also been demonstrated histologically with improved protection against oxidative stress and increased survival of motor neurons (20,21).
Below we will discuss some of the targets in our current research. We aim to improve ischemic tolerance and alter excitotoxity through two different models: (I) an in-vivo murine spinal cord ischemia-reperfusion model and the impact on motor outcomes and (II) through culturing murine spinal cord neurons in-vitro with exposure to oxygen-glucose deprivation followed by re-exposure to a normally oxygenated and glucose rich medium.
Modification of erythropoietin (EPO) receptor, and the signal transducer and activator of transcription (STAT) pathway
EPO is known to attenuate ischemic reperfusion injury in a variety of tissues through its anti-inflammatory and anti-apoptotic role in the setting of tissue injury, hypoxia and metabolic stress. In-vitro models have shown that EPO preserves neuron viability after oxygen-glucose deprivation. Furthermore, in animal models of ischemia-reperfusion, including our own, EPO has been shown to improve functional outcomes and reduce neuronal loss. The beta common receptor subunit of EPO receptor (βcR) is thought to play a critical role in the protective effects of EPO. The expression of the βcR subunit is upregulated in spinal cord tissue after ischemic exposure, and its neuronal preservation is lost in βcR viral-knockdowns (22,23). To optimize EPO protection, we sought to upregulate the expression of βcR.
Diazoxide (DZ), an activator of ATP-sensitive potassium (KATP) channels, is well recognized for mimicking cellular ischemia and its neuroprotective effects. Previously, it has been demonstrated that DZ induces βcR reception, with a peak at 36 hours in-vivo (24). We used DZ prior to ischemia and EPO at the time of ischemia to produce a synergistic effect, resulting in significantly preserved murine motor preservation compared to DZ alone, however, combination therapy did not increase expression of βcR (25). However, synergistic induction of DZ and EPO resulted in greatly increased expression of nerve growth factor (NGF), which is among the neurotrophins critical for development, maintenance and survival of the peripheral and central nervous systems (26).
We also sought to investigate other neuroprotective effects of DZ. Previously, DZ has also been shown to significantly increase tyrosine phosphorylation of STAT3, resulting in decreased apoptosis (27). In another mouse model of ischemia, we found that STAT3 was significantly upregulated by administration of DZ with preservation of motor function and histologically significant greater neuronal viability. Furthermore, the co-administration of LY5, a novel small-molecule STAT3 inhibitor, significantly inhibited STAT3 phosphorylation, with severe functional deficits by 12 hours and permanent paraplegia by 48 hours after reperfusion. Anti-B cell lymphoma 2 (BCL-2), a downstream agent in the STAT pathway, and a crucial anti-apoptotic inhibitor, was also significantly increased by DZ administration and abolished by co-administration with LY5 (28). Given the numerous mechanisms involved in the above agents, our future research hopes to better target individual elements of each pathway, and to improve our understanding of the function of STAT3.
Mitochondria: nitric oxide and KATP channel manipulation
Nicorandil is a vasodilator that acts through nitric oxide donation and KATP channel activation that has been used clinically for years outside of the United States to treat chronic angina (29-31). Previous studies have demonstrated that nicorandil has neuroprotective and cardioprotective effects in ischemic models and reduces ischemia-reperfusion injury via anti-apoptotic mechanisms. Administration ten minutes prior to ischemia-reperfusion in a rabbit model also resulted in improved motor function compared to an ischemic control (32). However, there was no data on optimal timing of administration or the exact mechanism of action underlying this finding. In a murine model, we induced SCI in mice via aortic cross-clamping and administered nicorandil prior to ischemia at various doses and time points. We successfully demonstrated that nicorandil pretreatment at 4 hours and 3 days prior to ischemia at any dose resulted in significant motor function preservation. At a histologic level, anterior horn neurons showed significant neuron preservation with nicorandil administration. Furthermore, we concomitantly administered mitochondrial KATP channel blocker, 5-hydroxy-decanoate (5HD), which resulted in complete abolishment of nicorandil’s beneficial effects (33). Our results suggested that the KATP channel activation was responsive for neuro-preservation, rather than vasodilation related to nitric oxide.
In another recent experiment, we co-administered a reactive oxygen species (ROS) scavenger which also resulted in obliteration of nicorandil’s beneficial effect. This suggests that downstream from KATP channel activation, the generation of ROS has a neuroprotective effect (34). In future studies, we hope to further clarify the role of nitric oxide independent of other ROS, and also to use a neuronal cell model to eliminate any possible role that might be played by KATP channel activation on other cell lines that are present in neuronal tissue and may contribute to our current results.
Ischemia-reperfusion injury in clinical practice
Although our focus has been on the above pharmacologic agents, many have been evaluated in an experimental setting for neuroprotective application. These include anti-inflammatories, antioxidants, anti-apoptotic agents and drugs that aim to mimic ischemic preconditioning, reduce metabolic demand, reduce excitotoxicity and osmotic agents to reduce tissue swelling. Many of these agents are difficult to apply clinically, as they have a myriad of other effects on other tissues, acting as both agonists and antagonists. Although in a controlled setting in the laboratory they may have a beneficial effect and although they may have theoretical and anecdotal benefits clinically, there is a paucity of randomized clinical trials to truly know their utility. Undoubtedly, ischemia-reperfusion injury plays a critical element in both the immediate and delayed presentation of spinal cord ischemia, and further investigation is of the utmost importance.
Other elements of clinical practice
Below we will discuss other elements of clinical practice that are frequently applied to mitigate the risk of SCI. In the immediate postoperative period, patients undergoing descending thoracic aneurysm (DTA)/thoracic aortic aneurysm (TAA) operations should be monitored in the intensive care unit with strict hemodynamic monitoring and maintenance, and hourly neurologic exams.
If concerns of injury arise, they should be quickly acted upon, through increased blood pressure goals, more aggressive cerebrospinal fluid (CSF) drainage as allowed by protocol and consideration of spinal imaging studies. Although concern for SCI is more apparent clinically, imaging studies can be a useful adjunct if there are concerns about lumbar drain placement, especially if there was a bloody tap upon placement, or if unilateral symptoms are present which are more likely to be epidural hematoma rather than true bilateral ischemic SCI. These aspects of care are further discussed below, in addition to discussion of other useful intraoperative adjuncts in the diagnosis and management of SCI.
Intraoperative neuromonitoring
Neurophysiologic intraoperative monitoring (NIOM) is often used as a guide to cerebral and spinal circulatory management. NIOM utilizes electroencephalography (EEG), peripheral somatosensory evoked potentials (SSEPs) and motor evoked potentials (MEPs) to detect neurologic deficits and possible malperfusion. When SCI does occur and is suggested by NIOM, most commonly it occurs with a change in MEP, followed by a potential SSEP change (35).
Neuromonitoring does face some criticism as its’ reliability is influenced by a number of factors including anesthesia, hypothermia, hypotension and surgical manipulation. Therefore, it can be difficult to determine if alerts correlate with true SCI. The perceived high false positive rate means that in a typical case, it is not uncommon to get short term alerts or loss of signal that can lead to unnecessary worry. Furthermore, although NIOM is exceptionally reliable with detecting immediate SCI, delayed SCI can occur without any neuromonitoring changes, with a recent study demonstrating up to 20% of delayed SCI occurring without any NIOM alert (35). Its utility is also limited by the fact it requires a multidisciplinary team for its use, which can be difficult to obtain in emergent cases, and at non-high volume aortic centers.
However, most current literature suggests that NIOM is reliable, including a meta-analysis by Tanaka et al. that found that MEPs has a summary sensitivity of 89.1% [95% confidence interval (CI): 47.9–98.6%] and a summary specificity of 99.3% (95% CI: 96.1–99.9%) for the detection of SCI (36). Furthermore, failure of MEPs to return post-operatively has an odds ratio of 15.87 for developing immediate spinal cord ischemia (35). Although literature focusing solely on SSEPs is sparse, SSEP changes alone typically do not correlate with SCI. However, they serve as a useful adjunct when paired with MEPs (35).
Importantly, significant advantages of neuromonitoring are that it can be used in anesthetized and sedated patients, and that when an alert does occur and it does seem to correlate with an intraoperative change, timely intervention can be performed. As a whole, neuromonitoring is a useful adjunct for recognition of possible SCI and allows for prompt intervention when necessary. As an institution, we take every alert seriously given the opportunity for timely intervention, and we believe that listening through the noise can have significant clinical benefit.
Hypothermia
Hypothermia is a powerful tool in increasing the interval of safe spinal cord ischemia. Hypothermia is thought to mitigate SCI through a number of factors, primarily through a reduction in cellular metabolic activity and oxidative demands, but also in reducing apoptotic activity, oxidative stress and inflammation (9). At 37 ℃, the maximum interval after which a full clinical recovery is possible is thought to be around 20 minutes. Cellular metabolic rate is reduced by slightly greater than a factor of two for every 10 ℃ of cooling. Thus, the safe window of ischemia is roughly doubled for every 10 ℃ drop in body temperature. Although profound hypothermia maximizes spinal cord protection, it poses other risks. Among these risks are pulmonary dysfunction and development of arrhythmias, but chief among them is the development of coagulopathy, which can be life threatening given the significant blood loss in some cases. A proposed adjunct to avoid these complications and allow for selective maximal cooling of the spinal cord is the infusion of 4 ℃ saline into the epidural space prior to cross clamping, however, there currently is insufficient evidence for this approach (37,38). Currently, no pharmacologic adjuncts can replicate the effects of hypothermia. Thus, most surgeons utilize cardiopulmonary bypass and circulatory arrest, with moderate hypothermia at 30 to 32 ℃, and some use of deeper hypothermia at 15 to 20 ℃ in more extensive aortic resections requiring longer ischemic times (39).
Preservation of distal perfusion
In order to perform open DTA/TAA repairs, mandatory cessation of SCBF is required due to aortic cross-clamping and circulatory arrest. SCBF below the level of T10 is completely absent when the aorta is clamped distal to the subclavian artery (9). In this situation, total ischemic time is of paramount importance and is directly correlated to the risk of developing SCI. However, this risk can be mitigated by establishing distal aortic flow through left heart bypass, which is associated with decreased incidence of adverse neurologic outcomes (40,41). Although left heart bypass allows for a lower activated clotting time and is preferred by some, we often use full bypass given it allows for the usage of pump suckers that can keep up with significant intraoperative blood loss. As an additional tool, when technically feasible, sequential aortic clamping can be used to maintain some level of perfusion and minimize ischemic burden (5).
Maintaining spinal perfusion
Given the significant role of SCBF in the development of SCI, optimizing spinal perfusion both intra-operatively and post-operatively is of critical importance. In addition to the other methods of minimizing ischemia duration and extent as discussed above, direct SA reimplantation can be considered to improve SCBF. Previously, before the broader focus on collateralization, SA reimplantation was believed to be the most effective approach to mitigating SCI. With more clinical experience, a more nuanced approach is necessary. If a SA is readily apparent, and it does not show any evidence of back bleeding, then reimplantation should be considered. If there is back bleeding, with reimplantation there is a risk of steal phenomenon that increases the likelihood of SCI, therefore, oversewing the SA would be the most protective approach (42). Furthermore, if SAs are not easily apparent, or if significant further dissection is needed to identify them that would prolong ischemia time, the risks of additional ischemia outweigh the benefit of reimplantation.
Optimizing systemic and intrathecal pressure to optimize SCPP are key adjuncts in both the intra-operative and post-operative setting. Augmenting systemic blood pressure for permissive hypertension after extensive SA sacrifice or coverage allows time for neo-vascularization to occur and for SCPP to return to optimal levels. Furthermore, when there is concern for developing SCI, there is good evidence for pressing systemic blood pressure intra- and post-operatively (6). Manipulating systemic blood pressure pharmacologically is a widely accepted strategy in the prevention of SCI. Additionally, consideration must be taken to central venous pressure, as in conjunction with CSF pressure they are to a degree additive to increasing outflow pressure, and thus strategies avoiding high venous pressure should be taken into consideration. Another more direct method of manipulating SCBF is through intrathecal administration of papaverine, which in a retrospective analysis of 398 patients demonstrated a significant reduction in incidence of paraplegia in those who received papaverine compared to those who did not (43).
CSF drainage improves SCBF by reducing intrathecal pressure, thus increasing SCPP. Placement of a CSF drain is not without significant risk, and thus it is typically employed prophylactically in patients at risk for SCI, or as a treatment method in cases with concern for developing SCI. In a meta-analysis of CSF drainage, Khan et al. demonstrated a significant reduction in SCI incidence in their pooled analysis, however, although the benefit remained clear for open TAA, results were conflicting regarding endovascular procedures (44). Most aortic centers recommend routine use of CSF drainage in high-risk patients and in open surgery, and to have a protocolized approach to management to optimize perfusion and avoid related complications.
Conclusions
The pathophysiology of SCI is complex and multifactorial, however great strides have been made in understanding and mitigating risk. Optimizing perfusion, whether directly, or through promoting collateralization, greatly reduces the risk of SCI and remains a focus of clinical research. Prevention of SCI requires an integrated, cohesive approach to maximize SCBF and promote ischemic tolerance, and our institutional approach and algorithm is summarized in Figure 4. In conclusion, it is of the utmost importance to continue to refine biochemical and clinical understanding of SCI, and to translate work at the bench into clinical practice.
Acknowledgments
Funding: None.
Footnote
Conflicts of Interest: The authors have no conflicts of interest to declare.
Open Access Statement: This is an Open Access article distributed in accordance with the Creative Commons Attribution-NonCommercial-NoDerivs 4.0 International License (CC BY-NC-ND 4.0), which permits the non-commercial replication and distribution of the article with the strict proviso that no changes or edits are made and the original work is properly cited (including links to both the formal publication through the relevant DOI and the license). See: https://creativecommons.org/licenses/by-nc-nd/4.0/.
References
- Ghincea CV, Ikeno Y, Aftab M, et al. Spinal Cord Protection for Thoracic Aortic Surgery: Bench to Bedside. Semin Thorac Cardiovasc Surg 2019;31:713-20. [Crossref] [PubMed]
- Scali ST, Giles KA, Wang GJ, et al. National incidence, mortality outcomes, and predictors of spinal cord ischemia after thoracic endovascular aortic repair. J Vasc Surg 2020;72:92-104. [Crossref] [PubMed]
- Hawkins RB, Mehaffey JH, Narahari AK, et al. Improved outcomes and value in staged hybrid extent II thoracoabdominal aortic aneurysm repair. J Vasc Surg 2017;66:1357-63. [Crossref] [PubMed]
- Keihanian F, Kouchakinejad-Eramsadati L, Yousefzadeh-Chabok S, et al. Burden in caregivers of spinal cord injury patients: a systematic review and meta-analysis. Acta Neurol Belg 2022;122:587-96. [Crossref] [PubMed]
- Parotto M, Ouzounian M, Djaiani G. Spinal Cord Protection in Elective Thoracoabdominal Aortic Procedures. J Cardiothorac Vasc Anesth 2019;33:200-8. [Crossref] [PubMed]
- Lindsay H, Srinivas C, Djaiani G. Neuroprotection during aortic surgery. Best Pract Res Clin Anaesthesiol 2016;30:283-303. [Crossref] [PubMed]
- Lindeire S, Hauser JM. Anatomy, back, artery of Adamkiewicz. In: StatPearls. Treasure Island (FL): StatPearls Publishing; July 25, 2022.
- Svensson LG, Crawford ES, Hess KR, et al. Experience with 1509 patients undergoing thoracoabdominal aortic operations. J Vasc Surg 1993;17:357-68; discussion 368-70.
- Griepp RB, Griepp EB. Spinal cord protection in surgical and endovascular repair of thoracoabdominal aortic disease. J Thorac Cardiovasc Surg 2015;149:S86-90. [Crossref] [PubMed]
- Bischoff MS, Brenner RM, Scheumann J, et al. Staged approach for spinal cord protection in hybrid thoracoabdominal aortic aneurysm repair. Ann Cardiothorac Surg 2012;1:325-8. [Crossref] [PubMed]
- Simon F, Wagenhäuser MU, Busch A, et al. Arteriogenesis of the Spinal Cord-The Network Challenge. Cells 2020;9:501. [Crossref] [PubMed]
- von Aspern K, Haunschild J, Ziemann M, et al. Evaluation of collateral network near-infrared spectroscopy during and after segmental artery occlusion in a chronic large animal model. J Thorac Cardiovasc Surg 2019;158:155-164.e5. [Crossref] [PubMed]
- Kasprzak PM, Gallis K, Cucuruz B, et al. Editor's choice--Temporary aneurysm sac perfusion as an adjunct for prevention of spinal cord ischemia after branched endovascular repair of thoracoabdominal aneurysms. Eur J Vasc Endovasc Surg 2014;48:258-65. [Crossref] [PubMed]
- Motyl CM, Beck AW. Strategies for prevention and treatment of spinal cord ischemia during F/BEVAR. Semin Vasc Surg 2022;35:297-305. [Crossref] [PubMed]
- Etz CD, Zoli S, Mueller CS, et al. Staged repair significantly reduces paraplegia rate after extensive thoracoabdominal aortic aneurysm repair. J Thorac Cardiovasc Surg 2010;139:1464-72. [Crossref] [PubMed]
- Branzan D, Etz CD, Moche M, et al. Ischaemic preconditioning of the spinal cord to prevent spinal cord ischaemia during endovascular repair of thoracoabdominal aortic aneurysm: first clinical experience. EuroIntervention 2018;14:828-35. [Crossref] [PubMed]
- Bell MT, Puskas F, Bennett DT, et al. Clinical indicators of paraplegia underplay universal spinal cord neuronal injury from transient aortic occlusion. Brain Res 2015;1618:55-60. [Crossref] [PubMed]
- Reece TB, Kern JA, Tribble CG, et al. The role of pharmacology in spinal cord protection during thoracic aortic reconstruction. Semin Thorac Cardiovasc Surg 2003;15:365-77. [Crossref] [PubMed]
- Smith PD, Puskas F, Meng X, et al. The evolution of chemokine release supports a bimodal mechanism of spinal cord ischemia and reperfusion injury. Circulation 2012;126:S110-7. [Crossref] [PubMed]
- Liang CL, Lu K, Liliang PC, et al. Ischemic preconditioning ameliorates spinal cord ischemia-reperfusion injury by triggering autoregulation. J Vasc Surg 2012;55:1116-23. [Crossref] [PubMed]
- Herajärvi J, Anttila T, Sarja H, et al. Exploring Spinal Cord Protection by Remote Ischemic Preconditioning: An Experimental Study. Ann Thorac Surg 2017;103:804-11. [Crossref] [PubMed]
- Foley LS, Fullerton DA, Bennett DT, et al. Spinal Cord Ischemia-Reperfusion Injury Induces Erythropoietin Receptor Expression. Ann Thorac Surg 2015;100:41-6; discussion 46. [Crossref] [PubMed]
- Foley LS, Fullerton DA, Mares J, et al. Erythropoietin's Beta Common Receptor Mediates Neuroprotection in Spinal Cord Neurons. Ann Thorac Surg 2017;104:1909-14. [Crossref] [PubMed]
- Yamanaka K, Eldeiry M, Aftab M, et al. Optimized induction of beta common receptor enhances the neuroprotective function of erythropoietin in spinal cord ischemic injury. J Thorac Cardiovasc Surg 2018;155:2505-16. [Crossref] [PubMed]
- Yamanaka K, Eldeiry M, Aftab M, et al. Synergistic Reduction of Apoptosis With Diazoxide and Erythropoietin in Spinal Cord Ischemic Injury. Ann Thorac Surg 2018;106:1751-8. [Crossref] [PubMed]
- Yamanaka K, Eldeiry M, Aftab M, et al. Synergetic Induction of NGF With Diazoxide and Erythropoietin Attenuates Spinal Cord Ischemic Injury. J Surg Res 2019;233:124-31. [Crossref] [PubMed]
- Hsieh YJ, Wakiyama H, Levitsky S, et al. Cardioplegia and diazoxide modulate STAT3 activation and DNA binding. Ann Thorac Surg 2007;84:1272-8. [Crossref] [PubMed]
- Yamanaka K, Eldeiry M, Aftab M, et al. Pretreatment With Diazoxide Attenuates Spinal Cord Ischemia-Reperfusion Injury Through Signaling Transducer and Activator of Transcription 3 Pathway. Ann Thorac Surg 2019;107:733-9. [Crossref] [PubMed]
- Tarkin JM, Kaski JC. Erratum to: Vasodilator Therapy: Nitrates and Nicorandil. Cardiovasc Drugs Ther 2017;31:483. [Crossref] [PubMed]
- Horinaka S, Yabe A, Yagi H, et al. Effects of nicorandil on cardiovascular events in patients with coronary artery disease in the Japanese Coronary Artery Disease (JCAD) study. Circ J 2010;74:503-9. [Crossref] [PubMed]
- Ishii H, Ichimiya S, Kanashiro M, et al. Effect of intravenous nicorandil and preexisting angina pectoris on short- and long-term outcomes in patients with a first ST-segment elevation acute myocardial infarction. Am J Cardiol 2007;99:1203-7. [Crossref] [PubMed]
- Wakamatsu Y, Shiiya N, Kunihara T, et al. The adenosine triphosphate-sensitive potassium channel opener nicorandil protects the ischemic rabbit spinal cord. J Thorac Cardiovasc Surg 2001;122:728-33. [Crossref] [PubMed]
- Ikeno Y, Ghincea CV, Roda GF, et al. Optimizing Nicorandil for Spinal Cord Protection in a Murine Model of Complex Aortic Intervention. Semin Thorac Cardiovasc Surg 2022;34:28-38. [Crossref] [PubMed]
- Ikeno Y, Ghincea CV, Roda GF, et al. Reactive Oxygen Species Mediate Nicorandil-induced Metabolic Tolerance to Spinal Cord Injury. Ann Thorac Surg 2021;112:38-44. [Crossref] [PubMed]
- Tanaka A, Nguyen H, Dhillon JS, et al. Reappraisal of the role of motor and somatosensory evoked potentials during open distal aortic repair. J Thorac Cardiovasc Surg 2021;S0022-5223(21)01231-9.
- Tanaka Y, Kawaguchi M, Noguchi Y, et al. Systematic review of motor evoked potentials monitoring during thoracic and thoracoabdominal aortic aneurysm open repair surgery: a diagnostic meta-analysis. J Anesth 2016;30:1037-50. [Crossref] [PubMed]
- Wynn MM, Acher CW. A modern theory of spinal cord ischemia/injury in thoracoabdominal aortic surgery and its implications for prevention of paralysis. J Cardiothorac Vasc Anesth 2014;28:1088-99. [Crossref] [PubMed]
- Shimizu H, Mori A, Yoshitake A, et al. Thoracic and thoracoabdominal aortic repair under regional spinal cord hypothermia. Eur J Cardiothorac Surg 2014;46:40-3. [Crossref] [PubMed]
- Griepp RB, Di Luozzo G. Hypothermia for aortic surgery. J Thorac Cardiovasc Surg 2013;145:S56-8. [Crossref] [PubMed]
- Coselli JS, LeMaire SA. Left heart bypass reduces paraplegia rates after thoracoabdominal aortic aneurysm repair. Ann Thorac Surg 1999;67:1931-4; discussion 1953-8. [Crossref] [PubMed]
- Estrera AL, Miller CC 3rd, Huynh TT, et al. Neurologic outcome after thoracic and thoracoabdominal aortic aneurysm repair. Ann Thorac Surg 2001;72:1225-30; discussion 1230-1. [Crossref] [PubMed]
- Etz CD, Weigang E, Hartert M, et al. Contemporary spinal cord protection during thoracic and thoracoabdominal aortic surgery and endovascular aortic repair: a position paper of the vascular domain of the European Association for Cardio-Thoracic Surgery†. Eur J Cardiothorac Surg 2015;47:943-57. [Crossref] [PubMed]
- Lima B, Nowicki ER, Blackstone EH, et al. Spinal cord protective strategies during descending and thoracoabdominal aortic aneurysm repair in the modern era: the role of intrathecal papaverine. J Thorac Cardiovasc Surg 2012;143:945-952.e1. [Crossref] [PubMed]
- Khan NR, Smalley Z, Nesvick CL, et al. The use of lumbar drains in preventing spinal cord injury following thoracoabdominal aortic aneurysm repair: an updated systematic review and meta-analysis. J Neurosurg Spine 2016;25:383-93. [Crossref] [PubMed]